Haas Ips Guide
- CNC Manual/Haas/Haas Programing/Haas Lathe Intuitive Programming Manual. Haas Lathe Intuitive Programming Manual. Views: 14482.
- The Haas Intuitive Programming System is a proprietary conversational programming system that uses easy-to-understand, step-by-step templates to guide the.
Haas Mill Intuitive Programming System (IPS) to easily program and. Write a complete program using all the programming instructions illustrated throughout.
Professional competency has traditionally been divided into 2 essential components: knowledge and skill. More recent definitions have recommended additional components such as communication, values, reasoning, and teamwork. A standard, widely accepted, comprehensive definition remains an elusive goal.
For infection preventionists (IPs), the requisite elements of competence are most often embedded in the IP position description, which may or may not reference national standards or guidelines. For this reason, there is widespread variation among these elements and the criteria they include.
As the demand for IP expertise continues to rapidly expand, the Association for Professionals in Infection Control and Epidemiology, Inc, made a strategic commitment to develop a conceptual model of IP competency that could be applicable in all practice settings. The model was designed to be used in combination with organizational training and evaluation tools already in place. Ideally, the Association for Professionals in Infection Control and Epidemiology, Inc, model will complement similar competency efforts undertaken in non-US countries and/or international organizations.
This conceptual model not only describes successful IP practice as it is today but is also meant to be forward thinking by emphasizing those areas that will be especially critical in the next 3 to 5 years. The paper also references a skill assessment resource developed by Community and Hospital Infection Control Association (CHICA)-Canada and a competency model developed by the Infection Prevention Society (IPS), which offer additional support of infection prevention as a global patient safety mission.
Open-Access: This article is an open-access article which was selected by an in-house editor and fully peer-reviewed by external reviewers. It is distributed in accordance with the Creative Commons Attribution Non Commercial (CC BY-NC 4.0) license, which permits others to distribute, remix, adapt, build upon this work non-commercially, and license their derivative works on different terms, provided the original work is properly cited and the use is non-commercial. See: http://creativecommons.org/licenses/by-nc/4.0/. The recent approval of gene therapy products in Europe and Asia and the upsurge of gene therapy products in clinical trials signal the rebound of this technology not only for many orphan diseases but also for non-life threatening diseases. Following the success of induced pluripotent stem (iPS) cells in research, other modified ex vivo gene therapies are also knocking on the door of the clinic. Historically, gene therapy has experienced many ups and downs and still faces many challenges. During the past 10 years, many new ideas have been tried, and the goal of making this technology a more effective treatment modality through greater safety and control is coming within reach.
The first clinical trial of iPS cells has begun, and cell mediated gene therapy products have reached phase III in some countries. The potential for tumorigenicity and immunogenicity are still concerns with these products, so physicians should understand the biological aspects of engineered cells in the clinic. In this review article, we attempted to provide a summary update of the current state of knowledge regarding this technology: that is, we reviewed products that have finished clinical trials, are still in clinical trials and/or are at the research stage. We also focused on the challenges, future directions, and strategies for making this technology available in the clinic. In addition, the available measures for making gene therapy products safer are within the scope of this article. It is also important to understand the manufacturing process for gene therapy products, because cell characteristics can change during the cell expansion process. When physicians use gene therapy products in the clinic, they should be aware of the viability, temperature sensitivity and stability of these cells because biologic products are different from chemical products.
Although we may not be able to answer all possible questions and concerns, we believe that this is the right time for physicians to increase their interest in and understanding of this evolving technology. Core tip: In this review article, the authors attempted to provide an up to date summary of the current knowledge regarding cell mediated gene therapy that is, we reviewed products that have finished clinical trial, are in clinical trial and at the research stage. The authors also tried to cover the challenges, future directions, and strategies to make this technology available in the clinic. This is the right time for the physicians to have knowledge of this evolving technology that already reached the bedside. INTRODUCTION When the first gene therapy trial of hematopoietic cells and lymphocytes was reported, it seemed that gene therapy might be the answer to treating most orphan diseases without major complications.
However, in early 2000, after the death of a patient during a gene therapy clinical trial, many researchers became very cautious about this new technology. The subsequent development of cancer as a result of ex vivo gene therapy treatment in 2003, made the regulatory authorities even more conservative. In 2009, after years of progress, the return of gene therapy was declared, and gene therapy was honored as “twenty-first century medicine”. Three products hit the market: p53-expressing Gendicine TM and Advexin TM, conditionally replicating adenovirus Oncorine TM and thymidine kinase + ganciclovir therapy, Cerepro TM8.
In 2012, it was reported that over 1800 gene therapy clinical trials had been completed in 31 countries. In addition, some countries’ governments have declared their intention to assist in the development of gene-related therapies at the federal government level.
More positive news for the field of gene therapy came with the award of the Nobel Prize for research on iPS cells in ex vivo gene therapy. This technology has produced a seismic shift in stem cell research and resulted in an increase in research for disease modeling of iPS cells. The ultimate goal of these studies is the treatment of diseases that have not been treatable by conventional methods. Safety issues, manufacturing issues and product quality issues need to be addressed before these products become available in the clinic. Genetic engineering can make the cells more vulnerable to cancer development and therefore alternative engineering methods are needed.
To address these safety concerns, many papers have been published and several clinical trials have been performed to develop gene therapy technology for diseases in which there is no current available treatment. Recently, the first clinical trial of iPS cells started in Japan, and ex vivo gene therapy for degenerative arthritis has reached phase III clinical trials.
In this review, we will discuss the status of cell mediated ( ex vivo) gene therapy, including clinical trials, safety issues, and manufacturing issues for clinical applications,. In the clinic, physicians should understand the characteristics of the treatments that they are using. Cell-related products are new to most doctors, and their use presents a challenge because these treatments were not available during most doctors’ medical school education and resident training programs.
In the area of regenerative medicine, these treatments cannot be confined to their own subspecialty. Understanding the mechanism of action of each product at the molecular level is necessary for doctors.
We acknowledge that doctors can resist change, but we believe that understanding molecular medicine is similar to understanding new electronic technologies in everyday life. ENGINEERED CELLS FOR CLINICAL TRIAL AND RESEARCH The idea of multiple treatment modalities is familiar to physicians. For mesenchymal stem cell (MSC) differentiation, different combinations and effects of growth factors have been reported. For gene therapy, the integration of multiple genes into cells (transfection) has been studied in many laboratories. The observation that cells into which multiple genes have been integrated show the characteristics of embryonic stem cells (ESCs) was first reported by the Yamanaka group in Japan. This group inserted four factors, Oct3/4, Sox2, KLF4 and c-Myc, into fibroblasts, making them pluripotent.
They showed that the engineered cells were similar to ESCs in terms of morphology, proliferation, surface antigens, gene expression, epigenetic status of pluripotent cell-specific genes and telomerase activity. Furthermore, these cells could differentiate into all of the cell types of the three germ layers in vitro. Other researchers showed the regenerative potential of iPS cells, with their ability to differentiate into various cell types-. To improve the safety of treatment with these cells, other researchers induced the differentiation of these cells without viral vectors, and even with recombinant proteins. These strategies were reported to effectively eliminate any risk of modifying the target cell genome with exogenous genetic sequences. Consequently, the authors demonstrated the possibility of generating safer iPS cells.
However, the stability of these cells has not been reported. For clinical applications, the manufacturing process should produce batches of cells with the same characteristics. If the cells’ characteristics change in the presence of different concentrations of a protein, the manufacturing process is not validated for consistency. Safety analysis should also be performed prior to clinical trials. IPS cell-based therapies need to be thoroughly evaluated in pre-clinical animal models before they can be applied to human subjects,.
Since 1998, the Food and Drug Administration (FDA) has been regulating cell therapies, beginning with “Guidance for human somatic cell therapy and gene therapy”. In 2008, the FDA released the guidelines “Content and Review of Chemistry, Manufacturing, and Control (CMC) Information for Human Somatic Cell Therapy Investigational New Drug Applications (INDs)”. In 2011, they released the guidelines “Potency tests for cellular and gene therapy products”.
If cells are engineered to generate a product, the harvesting method (Good Tissue Practice), engineering method, and potency testing protocols should be clearly defined. From the beginning of the development process, the investigators should clearly understand the mechanism of action of the product to develop a manufacturing process with a method for harvesting cells and for testing them, to consistently produce well characterized, high-quality cell-based products. For example, Crook et al (2007) reported on the activities and requirements for producing cGMP hESC lines including the derivation, banking, and characterization of these cells. In 2014, Nature Medicine, in collaboration with the Volkswagen Foundation, organized a meeting with a panel of experts in regenerative medicine to identify the most pressing challenges, as well as to formulate the crucial strategies and stem cell concepts that could best help advance the field of translational regenerative medicine. The panel identified four major issues: first, harnessing the potential of endogenous stem cells; second, deciphering therapeutic reprogramming; third, meeting the challenges of cell integration and function; and fourth, removing roadblocks to the translation of stem cell therapies. We believe that these opinions summarize the present and future of cell mediated gene therapy. Researchers have begun to address these challenges.
In particular, one patient-specific pluripotent stem cell therapy has been reported to be safe with respect to immunogenicity and is nearing clinical trials. Neurodegenerative disorders are very interesting clinical targets for this technology. An enormous number of cell therapies have been tested worldwide for the treatment of rare diseases.
Regenerative medicine cannot be defined without MSCs and ESCs; however, gene therapy is also an important area in regenerative medicine. Many regenerative medicine companies have started up, and various umbrella organizations such as the Alliance for Regenerative Medicine have been formed. In 2009 and 2013, three very interesting studies were reported,.
These studies integrated a lentiviral vector into patient hematopoietic stem cells and showed promising clinical results for Wiskott-Aldrich syndrome and X-linked adrenoleukodystrophy. In 2013, another group reported promising clinical results using lentiviral ex vivo gene therapy for metachromatic leukodystrophy. These serial successes for rare diseases are a hallmark of this technology in the clinic. It is clear that this technology is the flag bearer for the future of treating rare diseases. It was a triumphant success to overcome the pessimistic environment that existed after leukemia developed in X-linked severe combined immunodeficiency (X-SCID) patients treated with retroviral gene therapy. In contrast to retroviral gene therapy, they extracted hematopoietic stem cells from a patient and transduced these cells with a lentiviral vector carrying specific genes (Wiskott-Aldrich gene, ABCD1 and ARSA), producing cell clones that did not carry integrations near oncogenes. Consistent with this, the authors did not observe evidence of clonal expansions in the patients for up to 20 to 32 mo after gene therapy treatment.
In 2007, induced pluripotent stem cell lines derived from human somatic cells were developed. In 2008, it was reported that to better understand amyotrophic lateral sclerosis (ALS) and to develop a treatment, induced pluripotent stem cells were generated from a patient with the disease. The authors generated iPS cells from an 82-year-old woman diagnosed with a familial form of ALS. These patient-specific iPS cells possessed properties of embryonic stem cells and were successfully directed to differentiate into motor neurons, the cell types destroyed in ALS.
In 2009, an animal study of treating hemophilia A with iPS cells was reported. The authors inserted the genes encoding 3 transcription factors, Oct4, Sox2 and Klf4, into somatic cells. The plasma FVIII levels in these mice increased to 8% to 12% of wild type, the hemophilia A phenotype was corrected. This study shows the possibility for future expansion of iPS cells into the clinic.
Haas Ips Training Video
Furthermore, Zhang et al differentiated iPS cells into mature pancreatic insulin-producing cells. This work not only provides a new model with which to study the mechanism of human pancreatic specialization and maturation in vitro but also enables the possibility of utilizing patient-specific iPS cells for the treatment of diabetes. Since the iPS cell researchers won the Nobel Prize, Japan has pioneered the clinical application of these cells in rare diseases. Previous success at treating degenerative disorders with gene therapy led researchers to pursue gene therapy for degenerative eye disorders,. In a proof-of-concept study, they showed that iPS cells developed a structured outer nuclear layer with complete inner and outer segments in 3D sheets. The authors also observed host-graft synaptic connections by immunohistochemistry. Eventually, they characterized and developed a human pluripotent stem cell-derived retinal pigment epithelium cell sheet for use in clinical trials.
A clinical trial on macular degeneration is ongoing in Japan,. In their research on retinitis pigmentosa, the authors generated patient-derived iPS cells that recapitulated the disease phenotype and expressed markers of cellular stress.
This research created the opportunity for understanding the disease by creating iPS cell models of degenerative disorders. Recent trends in iPS cell research have allowed a shift to using iPS cells to model disease. Degenerative disorders have been the main focus of this research. IPS cells use the same transcriptional network as ESCs to generate neuro-epithelia. IPS cells can be differentiated into functionally appropriate neuronal cell types over the same developmental time course as human ESCs in response to the same set of morphogens.
Therefore, researchers have suggested the possibility of employing human iPS cells in pathological studies, therapeutic screening and autologous cell transplantation. Glial progenitor cells have also been suggested to be useful for modeling neurological diseases. Chung et al exploited the mutational correction of iPS cells and conserved the proteotoxic mechanisms to reverse the phenotypic response to α-synuclein, a key protein involved in Parkinson’s disease,. In 2014, iPS cell-based in vitro modeling of cardiomyopathy was also reported. The authors combined patient-derived and genetically engineered iPS cells to elucidate the pathophysiology underlying the cardiomyopathy of Barth syndrome, a mitochondrial disorder caused by mutation of the gene encoding taffazzin (TAZ). Using Barth syndrome iPS-cell-derived cardiomyocytes, they defined metabolic, structural and functional abnormalities associated with TAZ mutation.
Another interesting study reported modeling the genetic risk of schizophrenia by using iPS cells. In addition, pathways that are disrupted in motor neurons in human ALS were also identified through the genetic correction of mutant SOD1. The authors combined the reprogramming and differentiation of iPS cells with genome engineering and RNA sequencing to define the transcriptional and functional changes that are induced in human motor neurons by mutant SOD1,. Human pluripotent stem cells hold great potential for regenerative medicine, but the available cell types have limitations. Recently, an international cell bank for iPS cells was created. In addition, Ma et al’s approach of genome-wide analysis may be the future of iPS cell characterization.
These authors examined the DNA methylation and transcriptome profiles of ESCs and somatic nuclear-transferred cells. They observed that the DNA methylation and transcriptome profiles of iPS cells retained residual DNA methylation patterns typical of parental somatic cells. Therefore, they concluded that human somatic cells can be accurately reprogrammed to pluripotency by somatic cell nuclear transfer and are therefore ideal for cell replacement therapy. This approach has opened an interesting field for the characterization of iPS cells in the future. A summary of selected clinical and preclinical research programs involving cell-mediated gene therapy is presented in Table.
Target disease Stage Gene modification Target cell Ref. SAFETY AND EFFICACY ISSUES The fact that chondrocytes can be induced to differentiate into fibroblasts during cell culture spurs questions about potential differentiation issues.
The continuation of the cell division process relies on the interaction of the cells with a microenvironment that consists of other cells and the extracellular matrix. Cells respond to a variety of growth factors. The autocrine and paracrine modes of cell stimulation are key elements in this process.
The differentiation of murine C3H10T1/2 mesenchymal cells into chondrocytes in response to bone morphogenic protein has been observed. Similar papers have reported on the differentiation of specific cells into cells with different characteristics. Dedifferentiation-associated changes in morphology and gene expression have also been reported in primary human chondrocytes in culture. These observations make the transforming growth factor-beta (TGF-β) superfamily proteins possible target modifiers of cartilage and bone formation. However, there is also a concern with regard to safety and efficacy because changes in other characteristics can change the properties of a cell therapy and therefore make the cells unacceptable as a treatment. With respect to efficacy, these findings brought forth the need to identify clear biomarkers to identify and characterize cells for potential transplantation. Some researchers previously thought that MSCs do not induce immunogenicity.
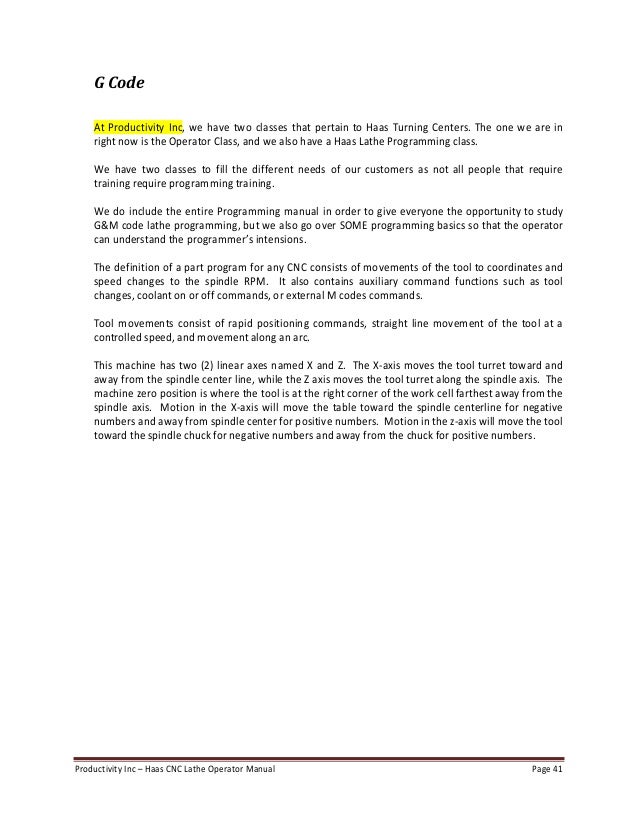
A report showing that human stem cells modulate the allogeneic immune response made people change their view about this concept. In 2005, several papers were published relating to the immunogenicity of mesenchymal stem cells. At this time, there was some confusion regarding the immunogenicity of mesenchymal stem cells. The T cell response to allogeneic human MSCs was evaluated for immunogenicity, tolerance and suppression. The authors concluded that MSCs can initiate the activation of T cells but do not elicit a T cell proliferative response because of an active suppression mechanism. These data were in support of Aggarwat and Pittenger’s work that was previously cited. However, somewhat contradictory data were also reported, namely that MSC immunogenicity was increased upon differentiation after transplantation into human and murine ischemic myocardium.
This paper indicated that differentiation of cells after injection into organs can cause problems, and the authors recommended a requirement for immunosuppressive therapy. They also indicated that allogeneic MSCs are not intrinsically immune privileged and that allogeneic MSCs can induce a T-cell response under appropriate conditions. In a pig study, intracardiac allogeneic porcine MSCs elicited an immune response despite their low immunogenic profile in vitro. This suggests that the in vivo characteristics of allogeneic MSCs might differ and emphasizes the importance of pursuing research both in vitro and in vivo. More specifically, allogeneic MSCs have been shown to induce immunogenicity, which limited their long term benefits for myocardial repair.
The authors of this study concluded that the long-term ability of allogeneic MSCs to preserve function in the infarcted heart is limited by a biphasic immune response whereby these cells transit from an immune privileged state to an immunogenic state after differentiation. We believe that not only the cell type but also the injection site is an important factor for immunogenicity.
To determine the potential for the biodistribution of gene-modified cells, the FDA recommends intravenous administration in an animal study to assess systemic distribution and persistence of the cells. Transduced genes can be the target for determining the distribution in cell-mediated gene therapy. After a single intravenous administration, the major organs should be checked to review the clearance of the cells for at least 30 d and for up to three months after injection.
At this stage of preclinical development, the maximum tolerated dose should be calculable. For safety and efficacy tests in animals, it is the standard practice to perform testing in two animal species. Clinical and histological analyses are included among the safety and efficacy tests, and treatment-related and dose-related toxicities can be assessed.
Single and multiple dose toxicity testing with up to a 1-year follow-up period may be required depending on the nature of the disease and the mode of treatment. The no-observed-adverse-effect level can be evaluated with these studies. This can be defined as the highest experimental point that is without adverse effects, although it does not address risk based on toxicologically relevant effects nor does it consider the progression of effects with respect to the duration or dose. In characterizing cell lines, the FDA may request tumorigenicity testing in accordance with the “Points to Consider in the Characterization of Cell Lines Used to Produce Biologicals” guidelines. Further studies of gene-modified cells may be recommended to assess the potential for these cells to adversely differentiate for 6 wk or up to 6 mo. Additional studies can be requested depending on the characteristics of the treatment modality. For allogeneic cells, studies to determine immunogenicity are required and may include assessing the HLA antigen expression by the cells, anti-HLA antibodies, the T cell response and multiple cytokines in animals and/or humans.
TUMORIGENICITY Karyotype analysis by using chromosome banding is the standard method for identifying numerical and structural chromosomal aberrations. A novel karyotyping technique, termed spectral karyotyping, was developed to increase the sensitivity of karyotyping. After reports of problems with viral vectors in gene therapy, this karyotyping issue becomes important in the cell-mediated gene therapy field. The FDA had concerns regarding injecting cells with chromosomal abnormalities in human clinical trials,. This concern arose as a result of the leukemia observed in the clinical trial of X-SCID patients. Oncogenesis or tumorigenicity has been considered a clinical hurdle for pluripotent stem cell therapies by certain authors,. These authors identified the seven risks of iPS cell therapy: integration of the gene into the host cell, chromosomal damage, clonal selection, incomplete programming, failure to silence pluripotent networks, DNA damage during cell culture and aberrant regulation of the imprinting process.
Adeno-associated virus was considered a relatively safe vector, but several authors still consider that this vector can induce chromosomal abnormalities,. For cells in which there is a karyotyping change after transfection, the FDA recommends rendering the cells replication incompetent ( e.g., via irradiation) prior to their being used in a clinical setting. In 2003, one of the most successful, problematic and influential cases of gene therapy was reported: LMO2-associated clonal T cell proliferation in two patients after gene therapy for SCID-X1,. The authors previously showed the correction of X-linked severe combined immunodeficiency in 9 out of 10 patients by using retrovirus-mediated gene transfer to autologous CD34 + bone marrow cells. However, 3 years after this gene therapy, uncontrolled, exponential clonal proliferation of mature T cells occurred in two patients. This incident was reported as an occurrence of leukemia following gene therapy.
Although these patients overcame the problem, the impact of this incident was sufficient to change the arena in terms of regulation, investment and research effort. Baum et al (2003) reviewed the side effects of this technology that are related to target cell manipulation, vector production, transgene insertion and expression, selection procedures for transgenic cells and immune surveillance. This unfortunate leukemic side effect of gene therapy can be used as a learning tool for developing safer, more effective gene therapies in the future. To overcome these potential side effects of gene therapy, many possible solutions have been devised,.
Chromosomal insulators, co-transfection of suicide genes under control of an inducible promoter, conditional expression of the transgene only in appropriate target cells, targeted transduction, cell type specific expression, targeted local administration, splitting of the viral genome, and site-specific insertion of the retroviral vector have all been proposed. A global iPS cell library has been proposed to preselect the donor genotype for immunological matching. This approach was proposed for immune compatibility, and similar approaches to reducing the potential for tumorigenicity can be devised. Individual cell lines that have undergone insertion site and gene expression analyses and have otherwise been evaluated for tumorigenicity and determined to be non-tumorigenic can be identified and used for other clinical trials. To reach this goal, more clinical and in vitro and in vivo non-clinical data should be generated.
MECHANISM OF ACTION AND BIOMARKER Some mesenchymal stem cell populations are relatively easy to harvest: for example, bone marrow MSCs. However, clinical application of these cells without preclinical evidence of efficacy and safety is unacceptable and will delay the development of clinically useful therapies. In particular, we need to better understand the mechanisms of action of stem cells after transplantation and learn how to control stem cell proliferation, survival, migration, and differentiation in pathological environments. Stem cell-based approaches have received much hype as potential treatments for neurodegenerative disorders. Indeed, they showed that transplantation of stem cells in an animal model of neurodegenerative disease can improve function by replacing lost neurons and glial cells and by mediating remyelination, trophic actions and modulation of inflammation. However, a clear understanding of the mechanism of action and a description of a biomarker for demonstrating efficacy should be devised for use in clinical trials.
Proposals submitted to the FDA for MSC-based products are undergoing a rapid expansion and are characterized by increased variability in donor and tissue sources, manufacturing processes, proposed functional mechanisms and characterization methods. Mendicino et al attempted to elucidate the FDA’s current perspective on the characterization of MSC-based products for clinical trials. The FDA proposed to characterize cell surface markers for IND applications of MSC-based products. Additionally, they found significant heterogeneity in the description of MSC bioactivity characterization in situations in which a candidate marker for a given assay has been defined. It remains unclear which particular set of markers will be sufficient to describe this complex and heterogeneous product class. Markers that can predict potential therapeutic benefit may allow the correlation of MSC characterization data with clinical data as they become available. For iPS cells, it can be more complicated.
IPS cells have been enthusiastically presented as a tool for aiding drug discovery by drug discoverers and commercial reagent and service providers alike. The future trend for research in this area relates to the maintenance of pluripotency and cellular reprogramming. To bring a product to the clinic, licensing, intellectual property and legal issues are also important. At this time, there may not be enough information for financial and business development professionals to evaluate the marketability of these products. Issues with developing cellular products and related assay development are only some of the challenges that are faced when integrating iPS cells into drug discovery. However, the potential for these cells to markedly improve the symptoms of patients motivates those involved in drug discovery to invest time and money to advance this technology.
MANUFACTURING OF CELL PRODUCTS One of the hurdles limiting the development of cellular therapies is the difficulty of cell expansion and the mass production required for a commercial product. The manufacturing technology for these products has developed in parallel with research on these products. Since the initial reports of the use of disposable bioreactors for cell culture that use wave-induced agitation, many innovations in single-use bioreactors have been reported. The technology ranges from 175 cm 2 tissue culture flasks to methods for scalable expansion of the cells. Routine commercial and clinical applications of human cells and their progeny require increasing cell quantities that cannot be provided by conventional adherent culture techniques.
Straightforward protocols for the expansion of undifferentiated ESC and iPS cells in suspension culture have been developed and reported. For ESCs, different methods for expansion to improve the culture conditions have been evaluated. A scalable GMP-compliant suspension system for human ESCs was reported by Chen et al,.
This suspension culture system provides a powerful approach for scaling-up the expansion of hESCs under defined, serum-free conditions for clinical and research applications. Disposable bioreactors are already widely accepted and in use for protein manufacturing,. Disposable components and systems are increasingly favored, both for improved process reliability and for the economic advantage they offer.
For this reason, many biotech producers of protein molecules are moving to disposable bioreactor modules that are pre-sterilized and meet the applicable regulatory requirements. In 2010, Eibl et al published a paper regarding disposable bioreactors, including the current state-of-the-art and recommended applications in biotechnology. In their paper, they provided a summary overview of the disposable bioreactors that were commercially available and described the domination of wave-mixed, orbitally shaken and stirred disposable bench top systems. They concluded that these novel systems are a viable alternative to traditional cell culture bioreactors at the bench top scale. In 1997, Genzyme gained approval for autologous chondrocyte transplantation. Although this technology was not a commercial success, it fueled the development of mass culture methods for chondrocytes. Not only have chondrocytes been used for the regeneration of cartilage in the knee of patients, but they have also been used for intervertebral disc regeneration.
To expand these cells for commercial usage, chondrocytes have been cultured and expanded in a microcarrier system,. They investigated human chondrocyte expansion in four macroporous gelatin microcarriers using two manufacturing processes that differed with respect to the amount of emulsifier used during the initial preparation and the gelatin cross-linking medium.
The authors observed a strong chondrocyte donor effect during the initial expansion phase. The final cell yield differed significantly between the microcarriers, and the result indicated that manufacturing differences affected chondrocyte densities.
For iPS cells, large-scale culture relies on the combined use of multiple growth components, including media containing various growth factors, extracellular matrices, 3D environmental cues and modes of multicellular association. Chen et al describe the criteria, considerations and suggestions for achieving optimal iPS cell growth.
RELEASE TEST AND QUALITY CONTROL As for chemical drugs, biological drugs should be consistent, active, pure, toxin-free and stable. Growth, harvesting and distribution into vials of cells should be conducted in a controlled GMP environment (Table ). Throughout the manufacturing process, in-process and release testing should be performed to ensure that each batch of product is safe and consistently meets the criteria for identity, purity and potency prior to administration to humans. The characteristics, viability and potency of the cells should be evaluated. The identity of the cells can be defined by morphologic examination and by markers of specific functions. For example, Type II collagen and GAG production are relevant criteria for cartilage cells.
Techniques such as immunohistochemical staining and RT-PCR are also good tools for this purpose. Cell potency can be measured by a quantitative analysis such as ELISA for specific therapeutic protein production, or by other product specific assays depending on the therapeutic mechanism. Cell viability and proliferation can be determined by specific staining ( e.g., tryptophan blue), MTT assay and/or by automated methods using fluoroscopy.
For gene-modified cells generated with a viral vector, replication-competent retrovirus detection may be required as a safety evaluation (Table ). IN THE CLINIC One of the first routes of delivery of cellular therapy was intra-articular because of some inherent advantages,. Some of the benefits of local delivery over systemic delivery include increased bioavailability, fewer adverse events, and lower total drug costs. Additionally, the intra-articular injection of cell therapies has advantages over intravenous administration in that systemic exposure is reduced and exposure to the antigen surveillance system is limited.
For these reasons, we have developed a cell mediated gene therapy for degenerative joint disease- and are currently conducting clinical trials with normal and engineered chondrocytes (called TG-C) injected into the knee joint for the treatment of osteoarthritis (Figure ). Figure 1 Schematic figure of TG-C manufacturing. WCB: Working cell bank. We are currently completing a Phase II study entitled ‘A Phase II Study to Determine the Efficacy and Safety of Allogeneic Human Chondrocytes Expressing TGF-β1 in Patients with Grade 3 Chronic Degenerative Joint Disease of the Knee’ in the United States. Phase III testing of this product is currently ongoing in South Korea.
To develop this product for clinical use, it was necessary to establish the stability of the product under frozen storage conditions and at room temperature for injection. The temperature of the product as it was transferred from manufacturing to storage to the clinic and within the clinic was carefully controlled and monitored. To date, more than 220 patients have been injected with TG-C. A schematic diagram of the manufacturing and injection processes is presented in Figure. The gene-modified cells in TG-C are irradiated to render them replication incompetent. These cells therefore produce the active TGF-β protein for only two weeks. These irradiated cells also produce Type-II collagen and glycosaminoglycan (GAG) by an autocrine mode of action and induce normal chondrocytes to produce Type II collagen and GAG by a paracrine mode of action in response to the TGF-β protein produced.
The releasing tests during the manufacturing of TG-C are described in Table. RCR: Replication competent retrovirus; LAL: Limulous amoebocyte lysate; RT-PCR: Reverse transcription polymerase chain reaction; CFR: Code of federal regulation; RAP: Rat antibody production. As TG-C is an allogeneic product; all patients in this study are being monitored for an immune response. To date, no immune response or serious adverse events attributable to TG-C have been observed. The decreased HLA-type antigenicity of the cells and the relatively immune privileged nature of the intra-articular injection site are thought to be contributing factors to the lack of immune response. CONCLUSION Our understanding of the nature of cell-based products and the diseases that they are intended to treat is being increased empirically: that is, through the scientific process of trial and error. Human clinical trials with cutting edge technology and novel products are part of that process and accordingly have their failures and successes, which are necessary if we are to learn and improve our understanding.
Industry’s perspective of cell-based therapy has changed in response to scientific developments and discoveries. Although there is a long way to go, a tremendous amount of clinical data have already been generated. In this review, we summarized the ongoing scientific effort to move cell mediated gene therapy into the clinic.
While some may still believe that such therapy is far from the clinic, it is our belief that now is the time for physicians to understand and embrace this new technology.